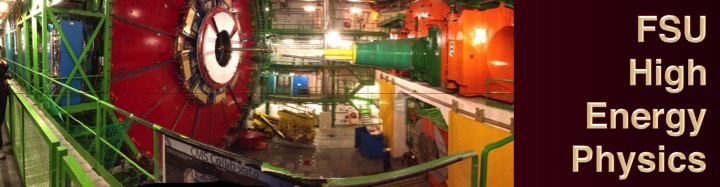
FSU Theoretical High Energy Physics
The FSU high energy theoretical physics group explores the fundamental laws that describe the fundamental constituents of matter and their interactions. See below for more about the work of Prof. Reina, Prof. Okui, Prof. Berg, and Prof. Owens (Emeritus).
Related Links
Prof. Reina's research centers on precision studies of the Standard Model of Particle Physics at colliders, with particular attention to the quantum effects of strong and electroweak interactions on rates and cross section of Standard Model (SM) processes. In recent years she has focused on the physics of the Large Hadron Collider (LHC) and aimed at advancing the state of the art of theoretical predictions of processes that could unveil new physics when compared with experimental measurements. Indeed, the potential of the LHC to discover new physics deeply hinges on the precise description of what we know and can predict within the SM itself.
Given the complexity of current high-energy experiments and the accuracy of experimental measurements, improving theoretical predictions requires the development of new approaches and sophisticated computational tools, together with a more precise description of the dynamics of high-energy collisions in different regimes and under different kinematical conditions. This is indeed the area of theoretical particle physics that has most contributed to fully exploit the potential of the LHC and allowed the discovery of the Higgs boson in 2012.
In this context Prof. Reina has spearheaded some important studies of the production of Higgs bosons and weak vector bosons in association with heavy quarks at hadron colliders and she has been focusing on the application and development of analytical and numerical algorithms for the systematic implementation of higher order perturbative field theory calculations. She has been an active member and convener of the Higgs Cross Section Working Group at CERN (https://twiki.cern.ch/twiki/bin/view/LHCPhysics/LHCHXSWG), and she has participated in the interplay between theorists and experimentalist during the exciting time of the Higgs boson discovery at the LHC.
The energy region explored by the LHC these days will provide crucial information on physics beyond the SM, either by direct (discovery of new particles) or by indirect evidence (effects on properties of SM particles). Even if no new particle is discovered at the LHC, we could have evidence of new physics from e.g. the deviation of the Higgs couplings from their SM pattern. Precision tests of the SM have been invaluable tools in the past to pinpoint the existence of new particles, from the top quark to the Higgs boson. In the same way, the measurement of the couplings of the discovered Higgs boson could become a main portal to new physics if results are interpreted in terms of non-SM effective interactions. Prof. Reina has been recently pushing this line of research individually and in the context of the HEPfit collaboration (http://hepfit.roma1.infn.it).
Prof. Okui explores models of physics that expand beyond the standard model of particle physics. Although the standard model (SM) of particle physics successfully describes all known subatomic phenomena down to the distance scale of about 0.00000001 times the size of the hydrogen atom, cosmological/astrophysical observations show that the SM is an incomplete theory. For example, we know that the particles of the SM only account for 4% of the mass of the present universe (but with a caveat to be explained below). 23% is called dark matter, some form of matter that interacts with us extremely feebly, hence appearing "dark" to us. Since there is no SM particle that has the right properties to be dark matter, this is a compelling experimental evidence that the SM is missing something. 73% is the energy of the vacuum itself, called dark energy. Dark energy could be accommodated in the SM, but only if we introduce an extremely tiny parameter, with 120 zeros after the decimal point. So this is crying out for a deep mechanism.
Actually, the SM can't even explain the 4% of "ordinary" matter. It is entirely made of matter, with a negligible antimatter component. While the SM is not perfectly symmetric between matter and antimatter, it can not explain this large of an effect. Besides these cosmological evidence against the SM, neutrino physics also tells us that the SM is incomplete. The SM predicts that neutrinos should be massless, but it has been known for longer than a decade that neutrinos must have mass, albeit tiny, to explain the firmly observed phenomenon called neutrino oscillation.
Motivated by these puzzles, Prof. Okui's research activities cover broad areas of physics beyond the SM such as collider phenomenology through model building to explore new experimental signatures and new observables at the LHC, dark matter model building and studying cosmological/astrophysical signals of dark matter, and building and using effective field theories to study subtle and interesting effects that are not obvious from standard perturbative calculations.
Prof. Berg works on Lattice Gauge Theory (LGT). Lattice regularization allows to calculate physical quantities that cannot be expanded in perturbation theory by means of computer simulations like Markov chain Monte Carlo (MCMC). An example is the mass spectrum of Quantum Chromodynamics (QCD).
To reach the continuum limit one has to send the lattice spacing to zero. One problem encountered is that of scale setting. One has to define a convenient, i.e., easy to calculate mass or length scale in which one expresses all other quantities. In a recent publication (Phys. Rev. D 95, 094508) we addressed this problem for pure SU(2) LGT, for which we were able to probe deep into the continuum limit thanks to massive parallel processing and CPU time funding by NERSC (National Energy Research Scientific Computing Center). For the first time we were able to control not only statistical, but also systematic errors, and they turned out to be dominant. Cooling length scales of the action were identified as efficient choices for setting the scale.
In another project we run simulations for the SU(2) Higgs model on the lattice. With the Higgs mass now experimentally known, it appears worthwhile to focus on investigating trajectories in the coupling constant space of the SU(2) Higgs model at which the Higgs to W mass ratio is kept at its physical value. Of major interest is whether or how far one can send the lattice spacing to zero along this line, measuring it in units of the inverse W mass. If this stops at a finite value, one obtains a scale at which the standard model becomes inconsistent and one may expect new physics. Such scales were previously set by estimating the so called Triviality limit of the model, where the Higgs becomes a free particle. With the low experimental Higgs mass value this triviality scale shifts all the way to the Planck scale. Consequently, the model should show a (pseudo) continuum limit behavior. However, our initial MCMC simulations appear to encounter a much smaller cut-off value, possibly due to interactions with the asymptotically free SU(2) part of the action. Eventually that could become a signal for new physics at an energy scale accessible by in the LHC.
Prof. Owens studies the theory of quantum chromodynamics (QCD). This is the theory that describes the interactions of quarks and gluons - particles that make up baryons (quark triplets) such as the neutron or proton and mesons (quark antiquark pair) such as the pion or rho. QCD can be used to describe how quarks and gluons scatter off each other in high energy collisions. However, at large particle accelerators the beams typically consist of protons or antiprotons. In order to apply QCD to such collisions, one needs to know how the momentum of the original particle is distributed over all of the quarks and gluons from which it is made. Parton Distribution Functions (PDFs) encode this information and are a necessary ingredient for making theoretical predictions for high energy accelerators such as the Large Hadron Collider. Some PDFs are known rather well, but others have rather large uncertainties. For example, a proton is composed of two u quarks, one d quark, and some quark-antiquark pairs all of which are held together by exchanges of gluons. The u PDF is rather well known as is the d PDF, at least for moderate momentum fractions. The u, d, and s antiquark distributions are less well known as is that of the gluon. At present we are unable to calculate the PDFs from first principles; they must be extracted from data. By examining many different types of high energy scattering processes one can determine what the underlying PDFs look like. Such a process is called a "global fit." Once the PDFs are known, one can make predictions for new processes and look for types of data that might not be well described by QCD.
Much of Prof. Owens' recent work has centered on PDF global fits. Specific topics of interest have included studies of the gluon distribution, the charm and bottom quark PDFs, and those of the strange quark and antiquark.
Prof. Owens is a founding member of the CTEQ Collaboration, a group of theorists and experimentalists devoted to studies of QCD. They also put on an annual summer school for advanced graduate students and postdocs.
We also have our hands in organizing conferences, physics schools,..: